Narrative review on alcoholic liver disease: from fibrosis to cancer
IntroductionOther Section
- Introduction
- Epidemiology
- Methods
table 2 )"> Risk factors for ALD and HCC (Table 2)- Mechanisms of alcohol-mediated fibrogenesis
- Mechanisms of alcohol-mediated hepatocarcinogenesis
- Summary, conclusion, and outlook
- Acknowledgments
- Footnote
- References
According to the WHO, chronic alcohol consumption is a risk factor for more than 200 diseases (1). Among them is alcoholic liver disease (ALD), one of the most prevalent liver diseases in Europe and the United States (2). Alcohol affects the liver in a typical sequence, starting from alcoholic fatty liver (AFL) to steatohepatitis, fibrosis, cirrhosis and finally hepatocellular cancer (HCC). However, alcohol is not only a hepatotoxin, but also a hepatocarcinogen. The fact that chronic alcohol consumption is associated with an increased risk also for extrahepatic organs, such as the upper alimentary and respiratory tract, the colorectum, and the female breast, demonstrates the specific carcinogenic effect of ethanol independent from liver cirrhosis (3). It is noteworthy that the administration of ethanol (5% in the drinking water) to B6C3F1 mice for more than two years resulted in a significant generation of hepatic adenomas and hepatocellular carcinomas without the administration of an additional carcinogen (4). The International Agency for Research on Cancer (IARC) in Lyon has classified ethanol as a class 1 carcinogen and has emphasized that acetaldehyde derived from ethanol metabolism is responsible at least for cancer of the esophagus (5).
For the liver, alcohol-driven fibrogenesis and additional specific mechanisms by which ethanol stimulates carcinogenesis act in concert to generate HCC. In this review, major focus has been laid on the role of alcohol in fibrogenesis, its role in cancer development and on unique mechanisms of alcohol, independent from cirrhosis, stimulating carcinogenesis. So far it is believed that, similar as in other liver diseases, hepatic fibrosis and cirrhosis are the predominate prerequisites for the development of HCC. We describe here additional important ethanol-specific mechanisms which may act in concert and add its carcinogenic potential not only with respect to ALD, but also with respect to other types of liver disease. For more detailed information on alcohol and HCC, it is referred to two excellent reviews published only recently (6,7).
In this review, publications from 1970–2021 have been considered with major emphasis on the past 20 years. All literature included was published in international journals in English, except for two summaries in the German language. This review includes all important research contributing to the current knowledge in the field.
We present the following article in accordance with the Narrative Review reporting checklist (available at https://dmr.amegroups.com/article/view/10.21037/dmr-21-91/rc).
EpidemiologyOther Section
- Introduction
- Epidemiology
- Methods
table 2 )"> Risk factors for ALD and HCC (Table 2)- Mechanisms of alcohol-mediated fibrogenesis
- Mechanisms of alcohol-mediated hepatocarcinogenesis
- Summary, conclusion, and outlook
- Acknowledgments
- Footnote
- References
According to the WHO report approximately 280 million individuals (4.1% of the population above 15 years of age) suffer from alcohol use disorder (AUD). Chronic harmful alcohol consumption results in approximately 3.3 million deaths every year (5.9% of all deaths) (7). ALD is the most relevant liver disease in Europe and in the United States. The Global Burden of Disease (GBD) project estimated 1.25 million deaths due to cirrhosis and chronic liver disease in 2016 worldwide (8). Approximately 30% are attributable to alcohol.
Worldwide, HCC is the second-highest cause of cancer-related death in men and the sixth highest in women. Of the 245,000 deaths caused by HCC, again in approximately 30 per cent alcohol was the major contributor (9).
Thus, unquestionable chronic alcohol consumption, defined as more than 40 grams of alcohol per day, consumed over years is not only one cause, it is the major cause for liver disease at least in Europe and in Germany.
For several years, it has been emphasized that non-alcoholic fatty liver disease (NAFLD) accounts for more deaths than ALD. However, it is difficult to separate these two entities since many individuals with NAFLD do drink additionally and it is well known that obese and overweight persons are especially prone to alcohol (10) as discussed below.
MethodsOther Section
- Introduction
- Epidemiology
- Methods
table 2 )"> Risk factors for ALD and HCC (Table 2)- Mechanisms of alcohol-mediated fibrogenesis
- Mechanisms of alcohol-mediated hepatocarcinogenesis
- Summary, conclusion, and outlook
- Acknowledgments
- Footnote
- References
This is a narrative review with a literature search on PubMed. The search was performed between August and November 2021 (Table 1).
Table 1
Items | Specification |
---|---|
Date of search | August–November 2021 |
Databases and other sources searched | PubMed |
Search terms used | Alcoholic liver fibrosis, alcohol and hepatocellular cancer, hepatocarcinogenesis |
Timeframe | 1970–2021 |
Inclusion and exclusion criteria | English language only, original studies and reviews only |
Selection process | HKS |
Any additional considerations, if applicable | Non-applicable |
HKS, Harvard Kennedy School.
Risk factors for ALD and HCC (Table 2)Other Section
- Introduction
- Epidemiology
- Methods
table 2 )"> Risk factors for ALD and HCC (Table 2)- Mechanisms of alcohol-mediated fibrogenesis
- Mechanisms of alcohol-mediated hepatocarcinogenesis
- Summary, conclusion, and outlook
- Acknowledgments
- Footnote
- References
Table 2
The quantity and time period of daily alcohol consumption |
Genetics |
Female gender |
Pre-existing other types of liver diseases |
Additional drug intake and exposure to certain xenobiotics |
Vitamin A or β-carotene in excess |
Smoking |
ALD, alcoholic liver disease.
The amount of alcohol
Since there is a linear dose-response relationship between alcohol consumption and the risk of cirrhosis and HCC, the amount of alcohol provides an indication for cirrhosis and HCC risk. Thus, >40 g per day over a sustained period of time (years) significantly increases the risk for advanced liver disease (11). The Dionysos study demonstrated a risk for ALD of approximately 7 for consumption of 50 grams alcohol per day and of 26 for consumption of 100 grams per day (12).
Genetics
The fact that only 10 to 20 per cent of individuals who drink heavily develop ALD suggests that individual risk factors exist. Clinicians knew that some patients with alcoholic cirrhosis reported a family history of ALD. Furthermore, monozygotic twins have a higher concordance rate for alcohol-related cirrhosis than dizygotic twins (13).
In the last years, the genetics on ALD became clearer. Several large, genome- wide association studies demonstrated that patatin-like phospholipase domain-containing protein 3 (PNPLA3) and, to a lesser extent, transmembrane 6 superfamily member 2 (TM6SF2) and membrane-bound O-acyltransferase domain-containing protein 7 (MBOAT7) are important genetic determinants of risk and severity of ALD (14-18). PNPLA3 is closely involved in lipid metabolism and is a risk factor for NAFLD and HCC. The mechanisms by which PNPLA3 influences the development of ALD are unclear. Mutation in TM6SF2 can result in hepatic fat accumulation due to a defect in the secretion of very-low-density lipoproteins, and mutation in MBOAT7 can affect the acetylation of phosphatidylinositol. Most recently, Stickel and his group identified the minor A allele in MARC1:rs2643438 as a gene loci decreasing the risk for alcoholic cirrhosis, while the minor C allele in HNRNPUL1:rs15052 increases the risk (18). MARC1 protein is involved in the detoxification of various xenobiotics including acetaldehyde and in the regulation of nitric oxide production, whereas HNRNPUL1, among others, can regulate transforming growth factor-β (TGF-β), a pro-fibrogenic cytokine.
Female gender
Women are more prone to alcohol compared to men, especially with respect to ALD (19). The reason for this is not clear, but may include:
- A lower first pass metabolism of alcohol in the stomach, leading to an increased absorption of alcohol (20,21). Thus, ethanol blood concentrations in women are significantly higher as those in men when the same amount of alcohol per kg body weight is consumed.
- A decrease in the water distribution space leading to higher alcohol levels as compared to men when the same amount of alcohol per kg body weight is ingested (22).
- An inhibition of estrogen degradation by alcohol resulting in higher blood estrogen concentrations (23-25). Estrogens may increase the uptake of endotoxins from the gut and may increase the sensitivity of Kupffer cells (KCs) to lipopolysaccharides (LPS) (26). In female patients more severe inflammatory response has been observed due to toll-like receptor (TLR4) signaling.
Pre-existing other types of liver disease
NAFLD and non-alcoholic steatohepatitis (NASH)
During the last years, one of the most important questions was raised: whether patients with NAFLD are allowed to drink alcohol. Data from epidemiology, clinical trials and experimental approaches have been somehow controversial. Therefore, this issue is discussed here in more detail.
Despite the fact that the pathogeneses of ALD and NAFLD share similarities, the mechanisms for non-alcoholic- (NAFL) and AFL are different (27). As alcohol consumption and an excess of dietary caloric intake may occur together, the effect of chronic alcohol consumption on patients with obesity and patients with NAFLD is of special interest. Various epidemiological studies report that >40 grams of alcohol per day and even moderate (20–40 g of alcohol per day) alcohol consumption can enhance hepatic steatosis, inflammation, fibrosis, and cirrhosis in patients who are overweight or obese (28). Unquestionable, obesity is a risk factor for ALD (11,28).
It was believed for a long time that moderate alcohol consumption has a beneficial effect in NAFLD. Epidemiological studies from Japan and Europe suggest that moderate alcohol consumption improves hepatic steatosis compared to non-drinkers due to an improvement of peripheral insulin resistance (29). Furthermore, various cross-sectional studies on NAFLD report a beneficial effect of alcohol consumption (>40 grams per day) on hepatic fat (30). Studies examining the effect of alcohol on histopathologically diagnosed NAFLD ended up with controversial findings. Some studies reported an enhanced fibrogenesis (31) and an elevation of serum transaminase activities (32) in patients with NAFLD when they consumed alcohol. Other studies, especially such with extremely overweighed patients, could not confirm this finding (30).
More recent studies report that 40 g alcohol and even moderate alcohol consumption of 20 to 40 g per day enhance hepatic steatosis, inflammation and fibrosis in overweight and obese patients (11,28,31-35). The Framingham-Heart-Study with 2,475 patients with fatty liver demonstrated that alcohol—even in low concentrations—is a risk factor for advanced liver disease in NAFLD (36). Another study from Japan found an accelerated fibrogenesis in moderate drinkers with NAFLD (37). A data analysis of the National Health and Nutrition Examination Survey III in the US could clearly demonstrate that more than 3 drinks per day significantly increase mortality in NAFLD patients (38). It is noteworthy that NAFLD patients with a fast ethanol metabolizing gene (ADH1B*2) have a significantly reduced risk for NASH and fibrosis. ADH1B*2 could modify the association between elevated body-mass index and the severity of NASH (39).
The data on alcohol and the development of HCC in patients who are overweight or obese and in patients with NAFLD patients is clearer. Almost all retrospective studies report an increased risk with alcohol consumption at any level for the development of HCC in patients with NASH (11,29,40,41).
In conclusion, considering all available data it seems difficult to analyze the role of moderate alcohol consumption in the progression of NAFLD. Various factors such as age, gender, ethnicity and genetics may modify this effect. In addition, whether alcohol is consumed in the presence of pure fatty liver without inflammation or when NASH is present. In clinical practice, it seems wise to recommend that at least patients with NASH should refrain from any amount of alcohol consumption.
Hepatitis B and C
While hepatitis B increases the risk of HCC five-fold when more than 30 g alcohol are consumed daily (11,42,43), it was shown for hepatitis C that people consuming 30–50 g alcohol per day have a risk of advanced fibrosis increased four-fold (11,43,44) and an even higher risk of HCC. Even smallest amounts of alcohol should be avoided by hepatitis C patients (45).
Hemochromatosis
Patients with hereditary hemochromatosis have an increased risk for ALD. The fact that patients with alcohol-induced HCC show an increased frequency of the heterozygosity of the hemochromatosis gene C282Y allele compared to HCC patients with a non-alcohol induced cirrhosis demonstrates that iron metabolism plays a role in alcohol-mediated hepatocarcinogenesis (46).
α1-antitrypsin deficiency
Individuals with heterozygous α1-antitrypsin deficiency also have an increased risk for ALD (47).
Drugs and xenobiotics
Many drugs interact with alcohol, even when alcohol is consumed in low quantities. This is predominantly relevant for centrally acting drugs, since the interaction between alcohol and these drugs can produce severe complications including death. In addition, the intake of paracetamol and isoniazid may lead to severe liver damage due to the generation of highly toxic intermediates via cytochrome P4502E1 (CYP2E1). Methotrexate and alcohol have a synergistic effect in the development of hepatic fibrosis. In this context it is referred to more detailed literature (48).
β-carotene and vitamin A
Chronic alcohol consumption induces CYP2E1, which is responsible for the degradation of retinol and retinoic acid (RA) to polar apoptotic metabolites (49-51). Although the loss of RA has deleterious consequences in cell differentiation and cancer development, substitution of RA, retinol or β-carotene further enhances the generation of these apoptotic metabolites resulting in liver damage (51-53).
Smoking
Smoking of more than 20 cigarettes per day increases the risk for ALD three-fold (54).
Mechanisms of alcohol-mediated fibrogenesisOther Section
- Introduction
- Epidemiology
- Methods
table 2 )"> Risk factors for ALD and HCC (Table 2)- Mechanisms of alcohol-mediated fibrogenesis
- Mechanisms of alcohol-mediated hepatocarcinogenesis
- Summary, conclusion, and outlook
- Acknowledgments
- Footnote
- References
As pointed out, cirrhosis of the liver is a prerequisite for the development of HCC in the patient with ALD. However, cases of HCC in ALD without cirrhosis have been reported. Thus, we will first discuss relevant mechanisms of hepatic fibrogenesis and the stimulating effect of ethanol, followed by the role of fibrosis in hepatocarcinogenesis, and finally particular alcohol-mediated mechanisms of hepatocarcinogenesis independent of cirrhosis.
Simplified mechanisms of hepatic fibrogenesis
Before discussing the effect of ethanol on fibrogenesis, some major mechanisms of fibrosis development will be summarized (55-60).
Fibrosis starts when hepatic parenchyma is injured. HSCs are the predominant cells producing extracellular matrix (ECM). In addition, hepatic myofibroblasts may also occur from mesothelial cells and activated portal fibroblasts. Damage activated molecular patterns (DAMPs) from injured hepatocytes activate KCs which secrete pro-fibrogenic substances to activate HSCs. In addition, inflammatory cytokines, reactive oxygen species (ROS), and iron overload also activate HSCs. Quiescent HSCs become myofibroblasts, produce growth factors, and generate ECM. The activation of HSCs may occur directly through the death of hepatocytes and cholangiocytes or through a variety of cytokines from innate lymphoid cells, KCs, Th17 cells, and bone marrow-derived monocytes (55). In this context interleukin 17 (IL-17) is of special importance since it stimulates HSCs to secrete collagen type I, TGF-β through activating NFkB and STAT3 (56). IL-17 is elevated in ALD. Th17 cells also produce interleukin 22 (IL-22) (61). IL-22 stimulates carcinogenesis by activating STAT3 (62).
TGF-β produced by hepatic macrophages, KCs, and HSCs, is of considerable importance in hepatic fibrogenesis. After TGF-β binds to its receptor on HSCs, Smad-dependent and Smad-independent pathways are activated, leading to collagen synthesis (58,59). Furthermore, microRNAs (miRNA) also have an effect on HSCs by TGF-β. For example, miR-29 is down-regulated by TGF-β resulting in an up-regulation of ECM protein in HSCs (63). For more detailed information it is referred to various review articles (55-60).
HSCs can also be activated by platelet-derived growth factor (PDGF) from platelets, macrophages and myofibroblasts (64) and by ROS, which is generated through cytokines as well as necrotic and apoptotic hepatocytes (Figure 1) (65). ROS occurs during mitochondrial oxidation when electrons are transferred from NADPH to molecular oxygen. NADPH oxidases play an important role (66). ROS is also generated through cytochrome P4502E1 mediated ethanol oxidation in hepatocytes as well as in KCs (67) (more details below). Interestingly, ROS seems to increase TGF-β expression and TGF-β inhibits anti-oxidative enzymes (68,69).
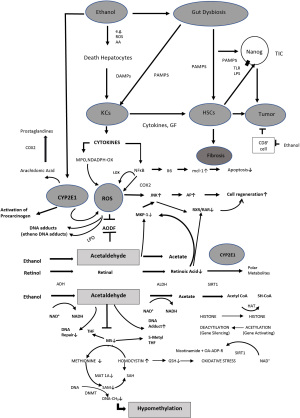
In addition, iron overload also results in oxidative stress and cellular damage through generation of ROS and is a major risk factor for a variety of liver diseases including ALD. The central regulator of iron homeostasis is hepcidin. Hepcidin inactivates the iron exporter ferroportin on hepatocytes, KCs, but also intestinal epithelial cells. In iron deficiency hepcidin is suppressed, in iron excess hepcidin is induced. The expression of hepcidin is influenced by a variety of factors such as Il-6, but also BMPs, Smads, hypoxia and ROS (70). Recently, it has been reported that hepcidin has anti-fibrotic effects (71). The complex relationship between all these factors is illustrated elsewhere (70,72).
Another mechanism promoting fibrogenesis is angiogenesis through inflammation-induced hypoxia. Activated HSCs produce vascular endothelial growth factor (VEGF) and angiopoietin-1 (73), and increase the expression of their receptors; on the other hand VEGF stimulates the proliferation of HSCs (74).
From inflammation to fibrosis: alcohol, cytokines, apoptosis, necroptosis, and pyroptosis
Alcohol can initiate hepatic fibrosis by directly stimulating HSCs or predominantly through inflammation. In humans, liver damage due to high alcohol consumption produces a cytokine storm syndrome characterized by the release of pro-inflammatory cytokines. The activation of the immune system is a host defense mechanism (75-77). In response to chronic, heavy alcohol exposure, hepatocytes express and secrete chemokines (78,79). The role of inflammation in chronic liver disease has also recently led to a new terminology called acute-on-chronic liver failure (ACLF). Although systemic inflammation is a hallmark of ACLF, its role in the development of this syndrome is poorly understood.
Exogenous inducers of cytokines include bacterial products from the gut called pathogen-associated molecular patters (PAMPs) and virulence factors. PAMPs elicit inflammation via innate pattern-recognition receptors (PRRs), whereas virulence factors generally trigger inflammation via functional feature identification. Endogenous inducers of cytokines include molecules released by necrotic cells and products of ECM breakdown, so-called damage-associated molecular patterns (DAMPs) (80-82). Death receptor-induced apoptosis in liver cells is mediated by both mitochondrial- and lysosomal permeabilization. Signaling between the endoplasmic reticulum and the mitochondria promotes hepatocyte apoptosis in response to excessive alcohol or free fatty acid generation during the metabolic process. Because apoptosis is a key feature of so many diseases of the liver, therapeutic modulation of liver cell death holds promise (83).
Necrosis in the liver cells is associated with acute injury. “Programmed” necrosis (necroptosis) has been described. Increasing evidence suggests an important role for hepatocyte apoptosis, necrosis, and necroptosis in the progression of ALD (83,84), although several other forms of cell death have been described, including pyroptosis, and autophagic cell death (75). Early during apoptosis, caspases are activated and cleave various substrates including cytokeratin 18 (CK18) (77,85). CK18 is a member of the intermediate filament family of cytoskeletal proteins. Cytokeratin-generated cleavage fragments of K18 can be detected in serum by the M30 antibody, which specifically labels early apoptotic fragments of cells (85).
An increase of apoptotic activity has been demonstrated in heavy drinkers undergoing alcohol detoxification. Apoptosis rate was highly associated with histological features of ballooning, lobular inflammation and fibrosis. These data suggested that alcohol has a strong inhibiting effect on cell regeneration and apoptosis while withdrawal will enhance cell regeneration (86-88).
In the recent study, we provide further evidence that M30 levels are generally higher in cirrhotic patients. The increase of M30 in response to withdrawal is only seen in non-cirrhotic patients. These novel findings suggest that patients with cirrhosis have a liver micro-environment that, independent of amount of alcohol consumption, causes continued cell death by apoptosis. Significantly elevated M65 levels in cirrhotic patients after detoxification confirm the continued hepatocyte death by necrosis. In contrast to apoptosis markers, TNF-α, IL-6, IL-8 and VEGF were only slightly elevated while no difference was seen with TGF-β in comparison to controls (88).
In summary, both apoptosis and necrosis play an important role in the development and progression of liver fibrosis (76).
Effect of ethanol on hepatic fibrogenesis
Alcohol-induced fibrogenesis actually starts following toxic and immunologic hepatocellular damage due to chronic alcohol consumption (11). The morphologic correlate is fatty degeneration, ballooning of the hepatocytes, apoptosis and necrosis. Stressed and dying hepatocytes produce signals; so-called DAMPs, which primarily induce the process of wound healing. Examples of these DAMPs are Hedgehog ligands which induce Hedgehog responsive genes Gli2, a-SMA and Vimentin in adjacent HSCs, thus initiate fibrogenic activation (89,90). Ethanol-mediated fibrogenesis starts around the central vein due to the fact that in this region ethanol metabolism is enhanced by alcohol dehydrogenase (ADH) and CYP2E1 (91). As a result, hepatocellular redox potential is enhanced, leading to more lactate, which is known to activate quiescent HSCs and oxidative stress via CYP2E1, more pronounced in the central area of the hepatic lobule as compared to the periphery. Thus, lactate, ROS, and acetaldehyde all activate HSCs (92-94).
The stress signals of damaged hepatocytes are processed by HSCs, hepatic endothelial cells of the sinusoids, KCs, dendritic cells and mast cells. The result is liberation of various inflammatory and fibrogenic messengers such as cytokines, chemokines and growth factors. As a result both inflammation and disposition of ECM with scaring and fibrosis occurs (11,55,60,95). It has been shown that miRNA play an important role in these pathophysiologic processes of the liver (96).
In addition to the stress signals of alcohol injured hepatocytes, another alcohol-associated effect on the progression of ALD is of importance. Ethanol leads to an increased uptake of endotoxins and LPS from the gut due to increased gut permeability (11,97-100). Estrogens seem to enhance gut leakiness (26). LPS acts as a signal molecule for TLR4-coupled signal pathways and initiates in HSCs and KCs especially the liberation of cytokines and chemokines which enhance inflammation, fibrogenesis and hepatocellular cell stress (101,102). Recent data underline the importance of the change in intestinal microbiota in the pathophysiology of ALD (103).
Besides the signals of ethanol stressed hepatocytes (DAMPs) and PAMPs direct effects of ethanol and acetaldehyde as well as the lipid-peroxidation products malondialdehyde or 4-hydoxynonenal on non-parenchymal cells have been described (104-106). They activate HSCs. Acetaldehyde is highly toxic and binds to proteins and DNA to form adducts, which can activate HSCs to secrete collagen type I and initiate KCs and endothelial cells to synthesize fibrogenic signals (see below).
Stimulation of HSCs by ethanol results—also through epigenetic mechanisms—in an enhanced expression of ECM (91,107). Acetaldehyde enhances the expression of pro-fibrogenic proteins such as collagen type I and fibronectin through protein kinase C-dependent signals in HSCs (108).
As pointed out, hepatic KCs are of major importance in sending inflammatory and fibrogenic signals through the binding of endotoxins to TLR4. KCs also contain CYP2E1 and are capable of oxidizing ethanol with the generation of ROS (67). A cross-talk between hepatocytes and KCs exists. Following ethanol exposure, hepatocytes secrete vesicles containing miRNA-122. This miRNA results in a much stronger reaction of KCs towards LPS. A miRNA-122-mediated up-regulation of HIF1a, a transcription factor which promotes tumor development, has been observed by alcohol (109-111).
Finally, ethanol decreases the anti-fibrotic effect of natural killer cells (NKCs) (112). NKCs secrete interferon gamma which kills activated HSCs (113,114). Similarly, the anti-fibrotic interleukin 22 (IL-22) is down-regulated in alcoholic hepatitis (115,116). Table 3 summarizes the effects of ethanol on fibrogenesis.
Table 3
(I) Alcohol alters the intestinal microbiota and results in an increased uptake of endotoxins from the gut due to increased gut permeability. Estrogens seem to enhance the gut leakiness. These endotoxins bind to toll-like receptor on Kupffer cells with the liberation of various cytokines and chemokines such as TGF-β1, IL-6, NFkB, TNF-α |
(II) Acetaldehyde as well as lactate, generated through the change in hepatic redox potential during ethanol oxidation, activates quiescent HSCs to become active myofibroblasts with the secretion of ECM proteins. This predominantly takes place around the central vein resulting in perivenular fibrosis |
(III) Alcohol and LPS induce hepatic fibrosis via activation of TGF-β signaling in a smad3-dependent fashion and down-regulation of smad7 |
(IV) Alcohol-mediated inflammation and CYP2E1 induction results in the generation of ROS stimulating fibrogenesis. Alcohol also results in hepatic iron overload favoring oxidative stress |
(V) Ethanol inhibits the anti-fibrotic effect of NKc |
(VI) Ethanol down-regulates IL 22, an antifibrotic cytokine |
(VII) Ethanol liberates miRNA-122, which sensitizes KCs towards LPS |
TGF-β, transforming growth factor β; IL-6, interleukin-6; NFkB, nuclear factor kappa-light-chain-enhanced of activated b-cells; TNF-α, tumor necrosis factor alpha; HSCs, hepatic stellate cells; ECM proteins, extra-cellular matrix proteins; LPS, lipopolysaccharides; CYP2E1, cytochrome P4502E1; ROS, reactive oxygen species; NKc, natural killer cells; IL 22, interleukin-22; miRNA-122, micro ribonucleic acid-122; KCs, Kupffer cells.
All these mechanisms contribute to the development of hepatic cirrhosis which has a variable prognosis depending on multiple factors. It was therefore important to development a histopathological score to predict prognosis. Most recently, Lackner and colleagues have presented a histological grading and staging system in ALD for the histological assessment of disease activity and fibrosis (117).
Fibrosis, alcohol and cancer
Since fibrosis is a dynamic process modulating tumor microenvironment, increasing deposition of collagen may modulate cellular signaling in all types of cells present in the liver, for example through binding specific receptors such as integrins and growth factor receptors (99,118,119). As already pointed out, during the process of fibrogenesis countless cytokines and chemokines are secreted from a variety of hepatic cells, mainly KCs and HSCs. Interestingly, alcohol sensitizes KCs via mechanisms dependent on endotoxins (120,121). HSCs initiate inflammation, proliferation, vascularization, and immunologic response, and some of them can act directly on tumor cells (HGF, TGF-β, PDGF, IL-6, Wnt ligands) favoring cell survival and growth (99,122-124).
Furthermore, HSCs can contribute to hepatocarcinogenesis by secreting VEGF, angiopoietin-1, and CXC chemokines important for tumor vascularization (99).
HSCs can also be activated by tumor-associated M2-polarized macrophages. Ectopic expression of TLR4 in hepatocytes may generate TLR4-NANOG-dependent liver tumor-initiating stem cell-like cells (TICs) after activation by LPS. TIC-mediated hepatocarcinogenesis and alcohol-mediated tumor development can be promoted through activated HSCs (125,126).
In addition, HSCs can also affect hepatic immunology (99) (see below).
In summary, HSCs activation is mandatory for fibrogenesis associated with carcinogenesis (127). It is important to note that alcohol-mediated fibrogenesis through HSCs activation is due to two major mechanisms: (I) CYP2E1 induction in hepatocytes resulting in ROS and activation of HSCs and (II) LPS from intestinal alcohol-mediated dysbiosis which also activate HCSs directly or through KCs via cytokines and chemokines. These two mechanisms are alcohol-specific and contribute to cancer via fibrosis (Figure 1).
Mechanisms of alcohol-mediated hepatocarcinogenesisOther Section
- Introduction
- Epidemiology
- Methods
table 2 )"> Risk factors for ALD and HCC (Table 2)- Mechanisms of alcohol-mediated fibrogenesis
- Mechanisms of alcohol-mediated hepatocarcinogenesis
- Summary, conclusion, and outlook
- Acknowledgments
- Footnote
- References
Beside the fact that alcoholic cirrhosis is the most important prerequisite for HCC, other alcohol-specific mechanisms play in concert and have an additional input into hepatocarcinogenesis (Table 4).
Table 4
Mechanism | Modes of action |
---|---|
(I) Mechanisms through inflammation and fibrogenesis | PAMPs from ethanol-induced gut dysbiosis and DAMPs from injured hepatocytes stimulate innate immunity and inflammation. Activated KCs secrete cytokines, chemokines and growth factors and activate HSCs. HSCs promote HCC formation via production of matrix or soluble factors which support tumor cell survival and growth. In inflammation, macrophages and PMNs generate ROS and tumor-associated M2 macrophages stimulate HSCs further |
Ectopic expression of TLR4 in hepatocytes and its activation by LPS induces HCC via generation of TLR4-NANOG-dependent liver TICs. In addition, activated HSCs also promote TIC-mediated alcohol-promoted liver tumor formation. | |
(II) Induction of CYP2E1 | CYP2E1 activates procarcinogens, degradates retinoids, and generates ROS leading to carcinogenic DNA adducts. Iron accumulation enhances ROS production further. Alcohol activates the canonical Wnt pathway, which may render β-catenin-dependent tumor growth and stimulate CYP2E1 transcription |
(III) Acetaldehyde | Acetaldehyde is a carcinogen, binds to proteins and DNA forming carcinogenic DNA adducts. Acetaldehyde also inhibits the anti-oxidative defense system and DNA repair |
(IV) Epigenetic modifications results in DNA and histone hypomethylation | |
(V) Immunosuppression | Alcohol consumption promotes HCC development via immunosuppression with reduced anti-tumor CD8+ cells and miRNA-122-mediated HIF-1α activation |
PAMPs, pathogen-associated molecular patterns; DAMPs, damage-associated molecular patterns; KCs, Kupffer cells; HSCs, hepatic stellate cells; HCC, hepatocellular cancer; PMNs, polymorphonuclear cells; ROS, reactive oxygen species; TLR4, toll-like receptor-4; LPS, lipopolysaccharides; TICs, tumor-initiating stem cell-like cells; CYP2E1, cytochrome P4502E1; miRNA-122, micro ribonucleic acid-122; HIF-1α, hypoxia-inducing factor alpha.
Acetaldehyde
Ethanol is a pro-carcinogen that requires its bioconversion to a primary carcinogenic metabolite, acetaldehyde. Acetaldehyde is electrophilic and forms adducts with DNA, and interstrand crosslinks. AA leads to point mutations at the hypoxanthine-guanine phosphoribosyl transferase (HPRT) locus on human lymphocytes, to micronuclei in eukaryotic cells, to sister chromatid exchange, to DNA strand breaks and to various chromosomal translocations. Further detectable cytotoxic effects of AA are a longer than normal cell cycle and an increased programmed apoptosis (128-130).
At molecular level, AA binds to cellular proteins and to DNA very quickly. Resulting AA adducts (such as the stable 1,N2-propanodeoxyguanosine adduct) are mutagenic and can further act as neo-antigens, which may in turn lead to the formation of specific antibodies with a respective immune reaction (131-134). Numerous in vitro and in vivo experiments in prokaryotic and eukaryotic cell cultures and in animals were able to prove direct mutagenic and carcinogenic characteristics. An evaluation by IARC classifies AA as a class I-carcinogen when combined with alcohol consumption (5).
Apart from these mutagenic and carcinogenic properties, AA also inhibits DNA repair mechanisms. An inhibiting effect on O6-methylguanine-DNA-transferase, an enzyme for DNA repair removing mutagenic adducts from the O6-binding site, has already been described for very low concentrations causing both genotoxicity and DNA repair failure (135).
Furthermore, acetaldehyde also inhibits the generation of the active methyl donor S-adenosyl methionine (SAMe) by inhibiting various enzymes involved in the generation of SAMe. As a result, a diminished availability of SAMe occurs with decreased methylation of DNA and histones supporting carcinogenesis (see below) (136).
Finally, as already pointed out, acetaldehyde also stimulates fibrogenesis by activating HSCs (92,137).
Cytochrome P4502E1 and oxidative stress
For almost 40 years, our laboratory has been studying the role of cytochrome P4502E1 (CYP2E1) in ALD and in alcohol-mediated carcinogenesis. Chronic alcohol consumption results in a significant increase of CYP2E1 (138). This induction is linked to an increased metabolism of alcohol to AA due to an increased activity of the microsomal ethanol-oxidizing system (MEOS) with CYP2E1 as an important component (139). Chronic drinkers show a CYP2E1 concentration in the liver that is up to ten times higher than that of non-drinkers (140). It was proven that the increase in CYP2E1 after alcohol consumption is due to a stabilization of CYP2E1 and not due to a de Novo synthesis (141).
In a series of experiments with human volunteers it could be shown that (I) already 40 g alcohol daily increases CYP2E1 significantly after one week (II) this CYP2E1 increase occurs already after 1 week of alcohol consumption and increases further over four weeks (III) the induction of CYP2E1 was heterogeneous. Although the majority of volunteers showed an induction, some did not (IV) CYP2E1 rapidly decreases after alcohol abstinence, within 2–3 days. However, the decrease to normal CYP2E1 levels was also found to be heterogeneous. The reason for these different reactions between individuals is not clear (142).
Since CYP2E1 is linked to various negative effects, these results could at least partly explain why only 40% of heavy drinkers suffer from alcoholic steatohepatitis and why only 10–20% suffers from cirrhosis (14-18). As discussed above, there are other genetic reasons why only a small percentage of heavy drinkers develop ALD.
A CYP2E1 induction in the colon mucosa was shown for alcohol patients suffering from liver diseases (143) and it was pointed out that the resulting oxidative stress leads to a damaged mucosa with increased penetration of endotoxins from the intestine to the portal vein system and the liver (144).
In the next paragraphs, the role of CYP2E1 in pro-carcinogen activation, retinoic acid degradation and ROS generation and its role in hepatocarcinogenesis will be discussed in more detail.
Activation of pro-carcinogens by CYP2E1
CYP2E1 not only catalyzes the oxidation of alcohol to AA via MEOS, but also the metabolism of various drugs, xenobiotics, and pro-carcinogens.
The first studies on the activation of carcinogens were already carried out in the 1970s and 1980s. These pioneer studies were able to show an increased activation of different pro-carcinogens, such as dimethylnitrosamine (DMN), benzo(a)pyrene and pyrolysates from amino acids and tobacco through hepatic and intestinal microsomes of rats after chronic alcohol consumption (145-147).
Since trace amounts of nitrosamines are present in some food and have been detected recently even in some drug preparation such as valsartan (148), the observation that ethanol administration results in an increased activity of hepatic DMN-demethylase with an increased capacity of liver microsomes to activate DMN in very low concentrations to a mutagen in the Ames test is of special interest (146). Moreover, an increased activation of DMN was also found in human liver microsomes of alcoholics (149).
Chronic alcohol ingestion not only increases the activation of nitrosamines, but also that of a variety of other pro-carcinogens, including compounds and components in tobacco smoke, such as benzo(a)pyrene, tobacco pyrolysate, tryptophan pyrolysate, but also a number of hepatotoxic substances, such as carbon tetrachloride, 2-aminofluoren, 2-acethylaminofluoren, 4-aminobiphenyl, benzene, cyclophosphamide, isoniazid, and methylazoxymethanol (147).
Loss of retinoic acid
Retinoic acid (RA), one of the most active forms of retinoids, is an important factor in the regulation of cell growth, apoptosis and cell differentiation. Reduced RA leads to uncontrolled cell proliferation, the loss of cell differentiation and dysregulated apoptosis, which might affect tumor promotion.
Chronic alcohol consumption leads to a significant decrease of hepatic retinol in patients with ALD (150). Using hepatic microsomes, it could be demonstrated that hepatic microsomes of rats, exposed to alcohol, showed an increased degradation of RA to polar RA metabolites such as 18-hydroxy-RS and 4-oxo-RS (50,51). RA metabolism in vitro was almost completely inhibited by clomethiazole (CMZ) and by CYP2E1 antibodies, leading to the conclusion that CYP2E1 is involved in RA metabolism (50). Rats which were chronically fed with alcohol showed a significantly lower RA concentration in the liver, related to an increased CYP2E1 activity. The hepatic RA values completely normalized after inhibition of CYP2E1 by CMZ as part of the diet (151). These results show that CMZ is able to normalize hepatic retinol and RA concentrations as well as RS by inhibiting their degradation through CYP2E1. CYP2E1 inhibition not only normalizes hepatic RA concentrations, but also other functions such as cell proliferation and cell cycle behavior (152).
We were able to show that alcohol-mediated CYP2E1 induction not only leads to a hepatic reduction of RA, but also to ROS production (see below). Both ROS and low RS values (via decreased MKP-1) result in an activation of the JNK pathway, in an increase in the transcription factor activator protein 1 (AP-1), and an increased c-fos and c-jun (14-fold increase in the liver of alcohol-fed animals in comparison to the control group) (153). At the same time, activation of the JNK metabolic pathway leads to a decreased expression of RA receptors RXR and RAR (there is a cross talk between the JNK pathway and RXR/RAR receptors) (49,53).
The overall effect of excessive alcohol consumption is a dysregulation of apoptosis, cell proliferation, immune function and inflammation. Inhibition of CYP2E1 cannot only reduce oxidative stress, but also restore normal retinoid signals and functions, which in turn might reduce the risk of cancer. Administering pure β-carotene, retinol or RA is dangerous, since these substances increasingly develop into toxic and apoptotic metabolites when CYP2E1 is induced (52,154). Thus, an administration of vitamin A or β-carotene together with alcohol leads to increased apoptosis and hepatocellular damage in the baboon (154).
Generation of ROS and exocyclic etheno-DNA adducts through alcohol metabolism via CYP2E1
Alcohol metabolism through CYP2E1 not only leads to the production of AA, but also to the generation of various ROSs, such as H2O2, OH- and carbohydrate-centered OH- (3,11,155). But as discussed above, ROS can also occur during inflammation via cytokines and chemokines. Normally, ROS are neutralized by a potent anti-oxidative defense system (AODS), regulated by the transcription factor Nrf2 (156). Chronic alcohol administration damages the AODS. Among others, AA binds to glutathione, an important radical scavenger. An increased production of ROS combined with a decreased detoxification has dramatic consequences:
ROS not only activates JNK and induces AP-1 leading to cellular hyperproliferation, a pro-carcinogenic status, but it also causes lipid peroxidation (LPO). LPO products such as malondialdehyde or 4-hydroxynonenal (4-HNE) are generated. 4-HNE binds to adenosine and cytosine, forming highly carcinogenic exocyclic etheno-DNA adducts such as 1,N6-etheno-2’deoxyadenosine (ƐdA) (157-164).
In a series of experiments investigating CYP2E1-overexpressing HepG2 cells, we were able to show that these ƐdA significantly correlate with CYP2E1 and 4-HNE. The adduct formation could be blocked by administering a specific CYP2E1 inhibitor, CMZ (165).
Furthermore, the role of CYP2E1 in ALE is impressively demonstrated in CYP2E1 knockout mice, not only showing a significant decrease of markers for oxidative stress after chronic alcohol consumption, but also demonstrating a highly-significant improvement of liver histology (166). On the other hand, animals over-expressing CYP2E1 show an increased severity of ALD (167,168). The suppression of CYP2E1 by CMZ, a specific CYP2E1 inhibitor, also improves ALD in animal experiments (169).
In order to investigate the effect of CYP2E1 on chemically-induced hepatocarcinogenesis, diethyl nitrosamine (DEN) was used for tumor induction. Over ten months, chronic alcohol administration led to the development of hepatocellular adenomas in DEN-induced rats, but not in the control group receiving the same amount of DEN (170). When CMZ was given to inhibit CYP2E1, the number of tumors was significantly reduced. After ten months, none of the animals which received DEN, alcohol and CMZ had an adenoma (170). Chronic alcohol administration led to a disturbed proliferation of normal hepatocytes in this animal model (171). It seems especially significant that alcohol increases the expression of TNF-α and that, at the same time, administration of CMZ reduces both the number of preneoplastic foci and the number of adenoma in our animal model. This convincingly underlines the role of CYP2E1 in carcinogenesis.
In addition, ethanol results in epigenetic changes associated with activation of canonical Wnt pathway (172). This may stimulate β-catenin-dependent tumor growth, but also CYP2E1 transcription (173).
Exocyclic etheno DNA-adducts could be detected in the livers of ALE patients and patients with other liver diseases associated with increased oxidative stress (163,174). CYP2E1, 4-HNE and ƐdA significantly correlated in liver biopsies of ALD patients (165). A more recent study with liver biopsies from almost 100 patients with ALD showed a highly significant correlation between CYP2E1 and ƐdA, as well as between CYP2E1 and the degree of liver fibrosis (174). These data show for the first time that CYP2E1 may also be responsible for the progression of liver fibrosis in ALE patients. Older cell culture studies, in which HSCs were co-incubated with CYP2E1 over-expressing HepG2 cells in the presence of alcohol, were able to show an increased activation of HSCs with increased fibrogenesis (94,137). ROS, generated by CYP2E1, was suggested as mechanism.
Finally, a recent randomized clinical trial convincingly demonstrated that the inhibition of CYP2E1 by CMZ over 10 days improved ALD significantly by αmobilizing hepatic fat and decreasing serum transaminase activities (175).
The role of CYP2E1 in ALD and alcohol-mediated cancer can be summarized as follows: (I) CYP2E1-driven ROS results in DNA damage, an increased fibrogenesis, and mechanisms involved in the pathogenesis of ALD and HCC; (II) CYP2E is also involved in the activation of various carcinogens and in the degradation of RA, which also contributes to cancer development.
The role of iron
The amount of iron in the liver is a prognostic factor for survival in ALD (176). Alcohol increases the intestinal absorption of iron with an increased depletion of iron in the liver, which may be responsible for DNA strand-breaks, p53 mutations and DNA adduct formation. Iron shows some toxicity with ROS resulting in hydroxyl radicals, which may react with various cellular structures. An important regulator of iron metabolism is hepcidin, which is synthesized in the liver and which inactivates the only iron export pump ferroportin located in the duodenum, but also in macrophages and the liver. An excess of iron induces hepcidin and a deficiency of iron leads to a suppression of hepcidin. In this situation ferroportin increases and pumps iron into the blood, which can then be used for erythropoiesis in the bone marrow. Hepcidin can be induced by hypoxia, cytokines such as Il6, LPS, and SMADs; it will be suppressed by ethanol, cell death, and TNF α, to name only a few factors (70,99,177).
Epigenetics
Epigenetic changes due to chronic ethanol consumption have a major influence on carcinogenesis. Alcohol can alter methylation and acetylation patterns of certain DNA regions as well as of histones. Alcohol also regulates miRNAs, an epigenetic mechanism that controls transcriptional events and the expression of certain genes (178).
Alcohol abuse causes aberrant DNA methylation, including genome-wide hypo-methylation caused by folate deficiency, a suppressed generation of methyl groups, and a reduced pool of the methyl-donor SAMe, leading to chromosomal instability (136). Hypo-methylation of promoters for oncogenes causes their aberrant activation and loss of imprinting, whereas hyper-methylation of promoters of genes involved in cellular differentiation or DNA repair promotes transformation. For more details it is referred to recent review articles (179,180).
The availability of the active methyl-donor SAMe is reduced by alcohol due to the inhibition of several different methyltransferase reactions. Ethanol inhibits methionine-adenosyl transferase which converts methionine into SAMe as well as enzymes that help regenerate methionine (betaine-homocysteine methyltransferase and methionine synthase). As a result, SAMe decreases and therefore DNA methylation also decreases (136). When SAMe is administered to animals, tumor formation induced by a carcinogen is inhibited (181). SAMe content in the liver is decreased in preneoplastic hepatic regions, and SAMe administration blocks the transformation of these lesions into cancer because of its DNA methylation capacity. Subsequently, SAME administration inhibits the expression of certain cancer-inducing genes such as c-myc, c-Ha-ras, and c-ka-ras (178).
Chronic alcohol consumption in general leads to DNA hypo-methylation. Beside the fact that alcohol decreases the availability of methyl groups, other factors may contribute to DNA hypo-methylation. ROSs produced by CYP2E1 are also involved in hepatic methylation patterns including DNA methylation (178). Thus, 8-hydroxy-2-deoxyguanosine decreases DNA methylation during DNA repair (182). DNA regions rich in the nucleosides cytosine and guanosine (i.e., CpG islands) may incorporate 8-OHdG, which inhibits the methylation of adjacent cytosine residues. The reason for this observation is an inhibition of methyltransferase, which results in DNA hypo-methylation (136). 8-OHdG formation can also interfere with a normal DNA methyltransferase function and prevent DNA re-methylation (182).
Histone modifications include methylation, acetylation, phosphorylation and ubiquitination. These modifications regulate gene expression, e.g. de-acetylation as well as hyper-methylation, associated with gene silencing and inactivation of genes suppressing tumor growth (tumor suppressor genes) with consecutive tumor promotion and stimulation of carcinogenesis [for more details see (178-180)].
In animal experiments, chronic alcohol ingestion increased acetylation of various histones (183). The nuclear level of β-catenin was increased indicating the activation of signaling pathway of canonical Wnt β-catenin pathway involved in tumor formation (178). In addition, it was shown that chronic alcohol-fed animals had an increased activity of histone acyltransferase (HAT) p300 (183). On the other hand, the activity of deacetylase (i.e., SIRT1) was also found to be increased after alcohol (178,183).
It is important to note that p21 expression is regulated by histone acetylation and is regulated by a protein complex that is associated with the p21 promoter including histone deacetylase1 which reduces acetylation (184). Thus, HDAC1 inhibitors induce p21-expression and cause cell cycle arrest (184,185). For a long a time it was not clear why liver cancer occurs more frequently in the livers of patients who abstained from alcohol for a longer period of time. However, one reason for that is the induction of p21 by alcohol with time after alcohol abstinence and that after that time cell cycle arrest disappears and hyper-proliferation occurs.
Effect of alcohol on the immune system
Alcohol suppresses the immune system and affects tumor surveillance. It has been shown that the spread of tumor metastases are facilitated when alcohol is given to experimental animals (186).
Alcohol decreases the number of anti-tumor CD8+ cells (187). Alcohol also results in a dysfunction of NKCs with a reduced cytotoxicity against cancer cells (112). The response of the innate immune system to inactivate transforming cells is promoted by chemokines and cytokines. Activated HSCs express factors that can affect activated T-cells which finally results in the protection of malignant cells from the immune system in an environment favoring survival and proliferation of pre-malignant cells (99).
Ethanol-mediated immune mechanisms are summarized in detail elsewhere (102).
Summary, conclusion, and outlookOther Section
- Introduction
- Epidemiology
- Methods
table 2 )"> Risk factors for ALD and HCC (Table 2)- Mechanisms of alcohol-mediated fibrogenesis
- Mechanisms of alcohol-mediated hepatocarcinogenesis
- Summary, conclusion, and outlook
- Acknowledgments
- Footnote
- References
The complex effects of ethanol on the development of fibrosis and cancer and their interaction is illustrated in Figure 1.
The major drivers of ethanol-mediated fibrogenesis and carcinogenesis are:
- Ethanol-induced hepatocellular damage with a cascade of signals (DAMPs) leading to hepatic inflammation and fibrosis;
- Ethanol-mediated gastrointestinal dysbiosis with increased uptake of endotoxins from an ethanol-induced leaky gut (DAMPs) resulting in KCs- and HSCs activation to secrete a variety of cytokines, chemokines and growth factors to stimulate fibrosis and inflammation, and;
- Ethanol-associated oxidative stress either due to inflammation or the induction of CYP2E1 leading to the generation of ROS which enhances fibrosis, but more importantly which results in protein- and DNA alterations.
In addition, ethanol results in epigenetic changes associated with activation of canonical Wnt pathway. This may stimulate β-catenin-dependent tumor growth, but also CYP2E1 transcription. Other factors include acetaldehyde, a loss of RA, generation of TICs and immunosuppression.
Thus, inhibition of HSCs activation on one hand and inhibition of CYP2E1 on the other hand may be two approaches to counteract fibrosis and cancer in ALD. Preliminary data show a beneficial effect of CYP2E1 inhibition in non-cirrhotic ALD even in humans by using the CYP2E1 inhibitor (162). Since CMZ cannot be administered for a longer period of time due to its addictive potential it is time to search for non-toxic CYP2E1 inhibitors.
Clinical implications deriving from the mechanisms discussed above may include approaches to prevent and to treat ALD. With respect to prevention, unquestionable alcohol abstinence is the best way. However, in AUDs this is sometimes difficult to obtain and requires psychiatric couselling eventually flanked by pharmacotherapy with anti-graving drugs.
Another approach would be early detection of ALD and HCC. This includes screening examinations of the liver for ethanol toxicity (e.g., serum transaminase activity) and liver function (serum albumin, bilirubin, blood coagulation), sonography and if possible transient elastography:
The American Cancer Society (ACS) has suggested decreasing alcohol availability, to increase alcohol taxes and to ban alcohol commercials as a general measure to decrease alcohol mediated cancers. In addition, the ACS suggests developing clinical strategies to identify individuals with a high risk profile for alcohol associated cancer with consecutive treatment options (188).
AcknowledgmentsOther Section
- Introduction
- Epidemiology
- Methods
table 2 )"> Risk factors for ALD and HCC (Table 2)- Mechanisms of alcohol-mediated fibrogenesis
- Mechanisms of alcohol-mediated hepatocarcinogenesis
- Summary, conclusion, and outlook
- Acknowledgments
- Footnote
- References
The authors wish to thank Ms. Laura Ehrhard for typing the manuscript and Mrs. Heike Engel for the design of the figure.
Funding: None.
FootnoteOther Section
- Introduction
- Epidemiology
- Methods
table 2 )"> Risk factors for ALD and HCC (Table 2)- Mechanisms of alcohol-mediated fibrogenesis
- Mechanisms of alcohol-mediated hepatocarcinogenesis
- Summary, conclusion, and outlook
- Acknowledgments
- Footnote
- References
Provenance and Peer Review: This article was commissioned by the Guest Editor (Ralf Weiskirchen) for the series “The Pathogenesis of Hepatic Fibrosis: Basic Facts and Clinical Challenges” published in Digestive Medicine Research. The article has undergone external peer review.
Reporting Checklist: The authors have completed the Narrative Review reporting checklist. Available at https://dmr.amegroups.com/article/view/10.21037/dmr-21-91/rc
Conflicts of Interest: Both authors have completed the ICMJE uniform disclosure form (available at https://dmr.amegroups.com/article/view/10.21037/dmr-21-91/coif). The series “The Pathogenesis of Hepatic Fibrosis: Basic Facts and Clinical Challenges” was commissioned by the editorial office without any funding or sponsorship. The authors have no other conflicts of interest to declare.
Ethical Statement: The authors are accountable for all aspects of the work in ensuring that questions related to the accuracy or integrity of any part of the work are appropriately investigated and resolved.
Open Access Statement: This is an Open Access article distributed in accordance with the Creative Commons Attribution-NonCommercial-NoDerivs 4.0 International License (CC BY-NC-ND 4.0), which permits the non-commercial replication and distribution of the article with the strict proviso that no changes or edits are made and the original work is properly cited (including links to both the formal publication through the relevant DOI and the license). See: https://creativecommons.org/licenses/by-nc-nd/4.0/.
ReferencesOther Section
- Introduction
- Epidemiology
- Methods
table 2 )"> Risk factors for ALD and HCC (Table 2)- Mechanisms of alcohol-mediated fibrogenesis
- Mechanisms of alcohol-mediated hepatocarcinogenesis
- Summary, conclusion, and outlook
- Acknowledgments
- Footnote
- References
- WHO. Global Status Report on alcohol and health 2018.
- Blachier M, Leleu H, Peck-Radosavljevic M, et al. The burden of liver disease in Europe: a review of available epidemiological data. J Hepatol 2013;58:593-608. [Crossref] [PubMed]
- Seitz HK, Stickel F. Molecular mechanisms of alcohol-mediated carcinogenesis. Nat Rev Cancer 2007;7:599-612. [Crossref] [PubMed]
- Beland FA, Benson RW, Mellick PW, et al. Effect of ethanol on the tumorigenicity of urethane (ethyl carbamate) in B6C3F1 mice. Food Chem Toxicol 2005;43:1-19. [Crossref] [PubMed]
- Baan R, Straif K, Grosse Y, et al. Carcinogenicity of alcoholic beverages. Lancet Oncol 2007;8:292-3. [Crossref] [PubMed]
- Taniai M. Alcohol and hepatocarcinogenesis. Clin Mol Hepatol 2020;26:736-41. [Crossref] [PubMed]
- Matsushita H, Takaki A. Alcohol and hepatocellular carcinoma. BMJ Open Gastroenterol 2019;6:e000260. [Crossref] [PubMed]
- World Health Organization. Disease burden and mortality estimates: Cause specific mortality, 2000-2016. Geneva, Switzerland: WHO, 2018. Accessed: 08/13/2018. Available online: https://www.who.int/healthinfo/global_burden_disease/estimates/en/index1
- Torre LA, Bray F, Siegel RL, et al. Global cancer statistics, 2012. CA Cancer J Clin 2015;65:87-108. [Crossref] [PubMed]
- Whitfield JB, Masson S, Liangpunsakul S, et al. Obesity, Diabetes, Coffee, Tea, and Cannabis Use Alter Risk for Alcohol-Related Cirrhosis in 2 Large Cohorts of High-Risk Drinkers. Am J Gastroenterol 2021;116:106-15. [Crossref] [PubMed]
- Seitz HK, Bataller R, Cortez-Pinto H, et al. Alcoholic liver disease. Nat Rev Dis Primers 2018;4:16. [Crossref] [PubMed]
- Bellentani S, Tiribelli C. The spectrum of liver disease in the general population: lesson from the Dionysos study. J Hepatol 2001;35:531-7. [Crossref] [PubMed]
- Hrubec Z, Omenn GS. Evidence of genetic predisposition to alcoholic cirrhosis and psychosis: twin concordances for alcoholism and its biological end points by zygosity among male veterans. Alcohol Clin Exp Res 1981;5:207-15. [Crossref] [PubMed]
- Buch S, Stickel F, Trépo E, et al. A genome-wide association study confirms PNPLA3 and identifies TM6SF2 and MBOAT7 as risk loci for alcohol-related cirrhosis. Nat Genet 2015;47:1443-8. [Crossref] [PubMed]
- Stickel F, Moreno C, Hampe J, et al. The genetics of alcohol dependence and alcohol-related liver disease. J Hepatol 2017;66:195-211. [Crossref] [PubMed]
- Schwantes-An TH, Darley R, Mathurin PGenomALC Consortium, et al. Genome-wide association study and meta- analysis identifies novel risk factors for alcohol related liver cirrhosis. Hepatol 2021;73:1920-31. [Crossref]
- Whitfield JB, Schwantes-An TH, Darlay R, et al. A genetic risk score and diabetes predict development of alcohol-related cirrhosis in drinkers. J Hepatol 2022;76:275-82. [Crossref] [PubMed]
- Innes H, Buch S, Hutchinson S, et al. Genome-Wide Association Study for Alcohol-Related Cirrhosis Identifies Risk Loci in MARC1 and HNRNPUL1. Gastroenterology 2020;159:1276-1289.e7. [Crossref] [PubMed]
- Becker U, Deis A, Sørensen TI, et al. Prediction of risk of liver disease by alcohol intake, sex, and age: a prospective population study. Hepatology 1996;23:1025-9. [Crossref] [PubMed]
- Frezza M, di Padova C, Pozzato G, et al. High blood alcohol levels in women. The role of decreased gastric alcohol dehydrogenase activity and first-pass metabolism. N Engl J Med 1990;322:95-9. Erratum in: N Engl J Med 1990;323:553 Erratum in: N Engl J Med 1990;322:1540. [Crossref] [PubMed]
- High blood alcohol levels in women. N Engl J Med 1990;323:58-62. [Crossref] [PubMed]
- Shimizu I, Kamochi M, Yoshikawa H, et al. In: Shimizu I. editor. Trends in Alcoholic Liver Disease Research. Clinical and Scientific Aspects 2012:23-40.
- Coutelle C, Höhn B, Benesova M, et al. Risk factors in alcohol associated breast cancer: alcohol dehydrogenase polymorphism and estrogens. Int J Oncol 2004;25:1127-32. [PubMed]
- Reichman ME, Judd JT, Longcope C, et al. Effects of alcohol consumption on plasma and urinary hormone concentrations in premenopausal women. J Natl Cancer Inst 1993;85:722-7. [Crossref] [PubMed]
- Ginsburg ES, Mello NK, Mendelson JH, et al. Effect of alcohol ingestion on estrogens in postmenopausal women. JAMA 1996;276:1747-51. [Crossref] [PubMed]
- Enomoto N, Yamashina S, Schemmer P, et al. Estriol sensitizes rat Kupffer cells via gut-derived endotoxin. Am J Physiol 1999;277:G671-G677. [PubMed]
- Seitz HK, Mueller S. Nichtalkoholische (NAFLE) und alkoholische Lebererkrankung (ALE). CME Springer Verlag 2018;15:45-57.
- Naveau S, Giraud V, Borotto E, et al. Excess weight risk factor for alcoholic liver disease. Hepatology 1997;25:108-11. [Crossref] [PubMed]
- Seitz HK, Mueller S, Hellerbrand C, et al. Effect of chronic alcohol consumption on the development and progression of non-alcoholic fatty liver disease (NAFLD). Hepatobiliary Surg Nutr 2015;4:147-51. [PubMed]
- Boyle M, Masson S, Anstee QM. The bidirectional impacts of alcohol consumption and the metabolic syndrome: Cofactors for progressive fatty liver disease. J Hepatol 2018;68:251-67. [Crossref] [PubMed]
- Ekstedt M, Franzén LE, Holmqvist M, et al. Alcohol consumption is associated with progression of hepatic fibrosis in non-alcoholic fatty liver disease. Scand J Gastroenterol 2009;44:366-74. [Crossref] [PubMed]
- Ruhl CE, Everhart JE. Joint effects of body weight and alcohol on elevated serum alanine aminotransferase in the United States population. Clin Gastroenterol Hepatol 2005;3:1260-8. [Crossref] [PubMed]
- Alatalo PI, Koivisto HM, Hietala JP, et al. Effect of moderate alcohol consumption on liver enzymes increases with increasing body mass index. Am J Clin Nutr 2008;88:1097-103. [Crossref] [PubMed]
- Loomba R, Bettencourt R, Barrett-Connor E. Synergistic association between alcohol intake and body mass index with serum alanine and aspartate aminotransferase levels in older adults: the Rancho Bernardo Study. Aliment Pharmacol Ther 2009;30:1137-49. [Crossref] [PubMed]
- Raynard B, Balian A, Fallik D, et al. Risk factors of fibrosis in alcohol-induced liver disease. Hepatology 2002;35:635-8. [Crossref] [PubMed]
- Long MT, Massaro JM, Hoffmann U, et al. Alcohol Use Is Associated With Hepatic Steatosis Among Persons With Presumed Nonalcoholic Fatty Liver Disease. Clin Gastroenterol Hepatol 2020;18:1831-1841.e5. [Crossref] [PubMed]
- Kashiwagi K, Yamaguchi A, Shiba S, et al. Moderate alcohol consumption is not associated with subclinical cardiovascular damage but with hepatic fibrosis in non-alcoholic fatty liver disease. Alcohol 2020;89:1-7. [Crossref] [PubMed]
- Younossi ZM, Stepanova M, Ong J, et al. Effects of Alcohol Consumption and Metabolic Syndrome on Mortality in Patients With Nonalcoholic and Alcohol-Related Fatty Liver Disease. Clin Gastroenterol Hepatol 2019;17:1625-1633.e1. [Crossref] [PubMed]
- Vilar-Gomez E, Sookoian S, Pirola CJ, et al. ADH1B*2 Is Associated With Reduced Severity of Nonalcoholic Fatty Liver Disease in Adults, Independent of Alcohol Consumption. Gastroenterology 2020;159:929-43. [Crossref] [PubMed]
- Ascha MS, Hanouneh IA, Lopez R, et al. The incidence and risk factors of hepatocellular carcinoma in patients with nonalcoholic steatohepatitis. Hepatology 2010;51:1972-8. [Crossref] [PubMed]
- Kawamura Y, Arase Y, Ikeda K, et al. Effects of Alcohol Consumption on Hepatocarcinogenesis in Japanese Patients With Fatty Liver Disease. Clin Gastroenterol Hepatol 2016;14:597-605. [Crossref] [PubMed]
- Chen CJ, Liang KY, Chang AS, et al. Effects of hepatitis B virus, alcohol drinking, cigarette smoking and familial tendency on hepatocellular carcinoma. Hepatology 1991;13:398-406. [Crossref] [PubMed]
- Morgan TR, Mandayam S, Jamal MM. Alcohol and hepatocellular carcinoma. Gastroenterology 2004;127:S87-96. [Crossref] [PubMed]
- Mueller S, Millonig G, Seitz HK. Alcoholic liver disease and hepatitis C: a frequently underestimated combination. World J Gastroenterol 2009;15:3462-71. [PubMed]
- Westin J, Lagging LM, Spak F, et al. Moderate alcohol intake increases fibrosis progression in untreated patients with hepatitis C virus infection. J Viral Hepat 2002;9:235-41. [Crossref] [PubMed]
- Fletcher LM, Powell LW. Hemochromatosis and alcoholic liver disease. Alcohol 2003;30:131-6. [Crossref] [PubMed]
- Strnad P, Buch S, Hamesch K, et al. Heterozygous carriage of the alpha1-antitrypsin Pi*Z variant increases the risk to develop liver cirrhosis. Gut 2019;68:1099-107. [Crossref] [PubMed]
- Seitz HK, Mueller S. Metabolism of alcohol and its consequences. Metabolism of Drugs and Xenobiotics 2012;493-516.
- Wang XD, Seitz HK. Alcohol and retinoid interaction. In: Watson RR, Preedy VR. editors. Nutrition and alcohol: linking nutrient interactions and dietary intake. CRC Press, Boca Raton, 2004:313-21.
- Liu C, Russell RM, Seitz HK, et al. Ethanol enhances retinoic acid metabolism into polar metabolites in rat liver via induction of cytochrome P4502E1. Gastroenterology 2001;120:179-89. [Crossref] [PubMed]
- Dan Z, Popov Y, Patsenker E, et al. Hepatotoxicity of alcohol-induced polar retinol metabolites involves apoptosis via loss of mitochondrial membrane potential. FASEB J 2005;19:845-7. [Crossref] [PubMed]
- Leo MA, Lieber CS. Hepatic fibrosis after long-term administration of ethanol and moderate vitamin A supplementation in the rat. Hepatology 1983;3:1-11. [Crossref] [PubMed]
- Seitz HK. Alcohol and retinoid metabolism. Gut 2000;47:748-50. [Crossref] [PubMed]
- Hagström H. Alcohol, smoking and the liver disease patient. Best Pract Res Clin Gastroenterol 2017;31:537-43. [Crossref] [PubMed]
- Dhar D, Baglieri J, Kisseleva T, et al. Mechanisms of liver fibrosis and its role in liver cancer. Exp Biol Med (Maywood) 2020;245:96-108. [Crossref] [PubMed]
- Meng F, Wang K, Aoyama T, et al. Interleukin-17 signaling in inflammatory, Kupffer cells, and hepatic stellate cells exacerbates liver fibrosis in mice. Gastroenterology 2012;143:765-776.e3. [Crossref] [PubMed]
- Bataller R, Brenner DA. Liver fibrosis. J Clin Invest 2005;115:209-18. [Crossref] [PubMed]
- Dooley S, ten Dijke P. TGF-β in progression of liver disease. Cell Tissue Res 2012;347:245-56. [Crossref] [PubMed]
- Dooley S, Meindl-Beinker NM, Breitkopf-Henlein K. Pathophysiologie der alkoholbedingten Fibrose und Zirrhose. In: Alkoholische Leber-und Krebserkrankungen. Seitz HK, Mueller S. editors. De Gruyter Verlag Berlin/Boston 2019:109-33.
- Lee YA, Wallace MC, Friedman SL. Pathobiology of liver fibrosis: a translational success story. Gut 2015;64:830-41. Erratum in: Gut 2015;64:1337. [Crossref] [PubMed]
- Kronenberger B, Rudloff I, Bachmann M, et al. Interleukin-22 predicts severity and death in advanced liver cirrhosis: a prospective cohort study. BMC Med 2012;10:102. [Crossref] [PubMed]
- Jiang R, Tan Z, Deng L, et al. Interleukin-22 promotes human hepatocellular carcinoma by activation of STAT3. Hepatology 2011;54:900-9. [Crossref] [PubMed]
- Roderburg C, Urban GW, Bettermann K, et al. Micro-RNA profiling reveals a role for miR-29 in human and murine liver fibrosis. Hepatology 2011;53:209-18. [Crossref] [PubMed]
- Pinzani M, Gesualdo L, Sabbah GM, et al. Effects of platelet-derived growth factor and other polypeptide mitogens on DNA synthesis and growth of cultured rat liver fat-storing cells. J Clin Invest 1989;84:1786-93. [Crossref] [PubMed]
- Luangmonkong T, Suriguga S, Mutsaers HAM, et al. Targeting Oxidative Stress for the Treatment of Liver Fibrosis. Rev Physiol Biochem Pharmacol 2018;175:71-102. [Crossref] [PubMed]
- Bedard K, Krause KH. The NOX family of ROS-generating NADPH oxidases: physiology and pathophysiology. Physiol Rev 2007;87:245-313. [Crossref] [PubMed]
- Koivisto T, Mishin VM, Mak KM, et al. Induction of cytochrome P4502E1 by ethanol in reat Kupffer cells. Alcohol Clin Exp Res 1996;20:207-12. [Crossref] [PubMed]
- De Bleser PJ, Xu G, Rombouts K, et al. Glutathione levels discriminate between oxidative stress and transforming growth factor-beta signaling in activated rat hepatic stellate cells. J Biol Chem 1999;274:33881-7. [Crossref] [PubMed]
- Barnes JL, Gorin Y. Myofibroblast differentiation during fibrosis: role of NAD(P)H oxidases. Kidney Int 2011;79:944-56. [Crossref] [PubMed]
- Mueller S, Rausch V. The role of iron in alcohol-mediated hepatocarcinogenesis. Adv Exp Med Biol 2015;815:89-112. [Crossref] [PubMed]
- Han CY, Koo JH, Kim SH, et al. Hepcidin inhibits Smad3 phosphorylation in hepatic stellate cells by impeding ferroportin-mediated regulation of Akt. Nat Commun 2016;7:13817. [Crossref] [PubMed]
- Silva I, Rausch V, Peccerella T, et al. Hypoxia enhances H2O2-mediated upregulation of hepcidin: Evidence for NOX4-mediated iron regulation. Redox Biol 2018;16:1-10. [Crossref] [PubMed]
- Taura K, De Minicis S, Seki E, et al. Hepatic stellate cells secrete angiopoietin 1 that induces angiogenesis in liver fibrosis. Gastroenterology 2008;135:1729-38. [Crossref] [PubMed]
- Kukla M. Angiogenesis: a phenomenon which aggravates chronic liver disease progression. Hepatol Int 2013;7:4-12. [Crossref] [PubMed]
- Neuman MG, Katz GG, Malkiewicz IM, et al. Alcoholic liver injury and apoptosis--synopsis of the symposium held at ESBRA 2001: 8th Congress of the European Society for Biomedical Research on Alcoholism, Paris, September 16, 2001. Alcohol 2002;28:117-28. [Crossref] [PubMed]
- Neuman MG. Cytokine - chemokines modulate fibrosis in alcoholic hepatitis. Rom J Hepatology 2007;3:19-34.
- Neuman MG, French SW, French BA, et al. Alcoholic and non-alcoholic steatohepatitis. Exp Mol Pathol 2014;97:492-510. [Crossref] [PubMed]
- Neuman MG, Schmilovitz-Weiss H, Hilzenrat N, et al. Markers of inflammation and fibrosis in alcoholic hepatitis and viral hepatitis C. Int J Hepatol 2012;2012:231210. [Crossref] [PubMed]
- Neuman MG, Brenner DA, Rehermann B, et al. Mechanisms of alcoholic liver disease: cytokines. Alcohol Clin Exp Res 2001;25:251S-3S. [Crossref] [PubMed]
- Neuman MG, Cohen L, Zakhari S, et al. Alcoholic liver disease: a synopsis of the Charles Lieber's Memorial Symposia 2009-2012. Alcohol Alcohol 2014;49:373-80. [Crossref] [PubMed]
- Molina PE, McClain C, Valla D, et al. Molecular pathology and clinical aspects of alcohol-induced tissue injury. Alcohol Clin Exp Res 2002;26:120-8. [Crossref] [PubMed]
- Guicciardi ME, Malhi H, Mott JL, et al. Apoptosis and necrosis in the liver. Compr Physiol 2013;3:977-1010. [Crossref] [PubMed]
- An P, Wei LL, Zhao S, et al. Hepatocyte mitochondria-derived danger signals directly activate hepatic stellate cells and drive progression of liver fibrosis. Nat Commun 2020;11:2362. [Crossref] [PubMed]
- Abdelmegeed MA, Banerjee A, Jang S, et al. CYP2E1 potentiates binge alcohol-induced gut leakiness, steatohepatitis, and apoptosis. Free Radic Biol Med 2013;65:1238-45. [Crossref] [PubMed]
- Neuman MG, French SW, Zakhari S, et al. Alcohol, microbiome, life style influence alcohol and non-alcoholic organ damage. Exp Mol Pathol 2017;102:162-80. [Crossref] [PubMed]
- Neuman MG, Malnick S, Maor Y, et al. Alcoholic liver disease: Clinical and translational research. Exp Mol Pathol 2015;99:596-610. [Crossref] [PubMed]
- Neuman MG, Seitz HK, French SW, et al. Alcoholic-Hepatitis, Links to Brain and Microbiome: Mechanisms, Clinical and Experimental Research. Biomedicines 2020;8:63. [Crossref] [PubMed]
- Neuman MG, Mueller J, Mueller S. Non-invasive Biomarkers of Liver Inflammation and Cell Death in Response to Alcohol Detoxification. Front Physiol 2021;12:678118. [Crossref] [PubMed]
- Rangwala F, Guy CD, Lu J, et al. Increased production of sonic hedgehog by ballooned hepatocytes. J Pathol 2011;224:401-10. [Crossref] [PubMed]
- Jung Y, Witek RP, Syn WK, et al. Signals from dying hepatocytes trigger growth of liver progenitors. Gut 2010;59:655-65. [Crossref] [PubMed]
- Lieber CS. Alcohol and fibrogenesis. Alcohol Alcohol Suppl 1991;1:339-44. [PubMed]
- Casini A, Cunningham M, Rojkind M, et al. Acetaldehyde increases procollagen type I and fibronectin gene transcription in cultured rat fat-storing cells through a protein synthesis-dependent mechanism. Hepatology 1991;13:758-65. [PubMed]
- Jauhonen P, Baraona E, Miyakawa H, et al. Mechanism for selective perivenular hepatotoxicity of ethanol. Alcohol Clin Exp Res 1982;6:350-7. [Crossref] [PubMed]
- Nieto N, Friedman SL, Cederbaum AI. Stimulation and proliferation of primary rat hepatic stellate cells by cytochrome P450 2E1-derived reactive oxygen species. Hepatology 2002;35:62-73. [Crossref] [PubMed]
- Krenkel O, Tacke F. Liver macrophages in tissue homeostasis and disease. Nat Rev Immunol 2017;17:306-21. [Crossref] [PubMed]
- Szabo G, Bala S. MicroRNAs in liver disease. Nat Rev Gastroenterol Hepatol 2013;10:542-52. [Crossref] [PubMed]
- Bjarnason I, Peters TJ, Wise RJ. The leaky gut of alcoholism: possible route of entry for toxic compounds. Lancet 1984;1:179-82. [Crossref] [PubMed]
- Nagy LE, Ding WX, Cresci G, et al. Linking Pathogenic Mechanisms of Alcoholic Liver Disease With Clinical Phenotypes. Gastroenterology 2016;150:1756-68. [Crossref] [PubMed]
- Boye A, Zou YH, Yang Y. Metabolic derivatives of alcohol and the molecular culprits of fibro-hepatocarcinogenesis: Allies or enemies?. World J Gastroenterol 2016;22:50-71. [Crossref] [PubMed]
- Thurman RG. II. Alcoholic liver injury involves activation of Kupffer cells by endotoxin. Am J Physiol 1998;275:G605-G611. [PubMed]
- Lackner C, Tiniakos D. Fibrosis and alcohol-related liver disease. J Hepatol 2019;70:294-304. [Crossref] [PubMed]
- Sutti S, Bruzzì S, Albano E. The role of immune mechanisms in alcoholic and nonalcoholic steatohepatitis: a 2015 update. Expert Rev Gastroenterol Hepatol 2016;10:243-53. [Crossref] [PubMed]
- Schnabl B, Brenner DA. Interactions between the intestinal microbiome and liver diseases. Gastroenterology 2014;146:1513-24. [Crossref] [PubMed]
- Kwon HJ, Won YS, Park O, et al. Aldehyde dehydrogenase 2 deficiency ameliorates alcoholic fatty liver but worsens liver inflammation and fibrosis in mice. Hepatology 2014;60:146-57. [Crossref] [PubMed]
- Setshedi M, Wands JR, Monte SM. Acetaldehyde adducts in alcoholic liver disease. Oxid Med Cell Longev 2010;3:178-85. [Crossref] [PubMed]
- Mello T, Ceni E, Surrenti C, et al. Alcohol induced hepatic fibrosis: role of acetaldehyde. Mol Aspects Med 2008;29:17-21. [Crossref] [PubMed]
- Page A, Paoli PP, Hill SJ, et al. Alcohol directly stimulates epigenetic modifications in hepatic stellate cells. J Hepatol 2015;62:388-97. [Crossref] [PubMed]
- Svegliati-Baroni G, Ridolfi F, Di Sario A, et al. Intracellular signaling pathways involved in acetaldehyde-induced collagen and fibronectin gene expression in human hepatic stellate cells. Hepatology 2001;33:1130-40. [Crossref] [PubMed]
- Lagos-Quintana M, Rauhut R, Yalcin A, et al. Identification of tissue-specific microRNAs from mouse. Curr Biol 2002;12:735-9. [Crossref] [PubMed]
- Momen-Heravi F, Bala S, Kodys K, et al. Exosomes derived from alcohol-treated hepatocytes horizontally transfer liver specific miRNA-122 and sensitize monocytes to LPS. Sci Rep 2015;5:9991. [Crossref] [PubMed]
- Ambade A, Satishchandran A, Szabo G. Alcoholic hepatitis accelerates early hepatobiliary cancer by increasing stemness and miR-122-mediated HIF-1α activation. Sci Rep 2016;6:21340. [Crossref] [PubMed]
- Jeong WI, Park O, Gao B. Abrogation of the antifibrotic effects of natural killer cells/interferon-gamma contributes to alcohol acceleration of liver fibrosis. Gastroenterology 2008;134:248-58. [Crossref] [PubMed]
- Radaeva S, Sun R, Jaruga B, et al. Natural killer cells ameliorate liver fibrosis by killing activated stellate cells in NKG2D-dependent and tumor necrosis factor-related apoptosis-inducing ligand-dependent manners. Gastroenterology 2006;130:435-52. [Crossref] [PubMed]
- Melhem A, Muhanna N, Bishara A, et al. Anti-fibrotic activity of NK cells in experimental liver injury through killing of activated HSC. J Hepatol 2006;45:60-71. [Crossref] [PubMed]
- Kong X, Feng D, Wang H, et al. Interleukin-22 induces hepatic stellate cell senescence and restricts liver fibrosis in mice. Hepatology 2012;56:1150-9. [Crossref] [PubMed]
- Ki SH, Park O, Zheng M, et al. Interleukin-22 treatment ameliorates alcoholic liver injury in a murine model of chronic-binge ethanol feeding: role of signal transducer and activator of transcription 3. Hepatology 2010;52:1291-300. [Crossref] [PubMed]
- Lackner C, Stauber RE, Davies S, et al. Development and prognostic relevance of a histologic grading and staging system for alcohol-related liver disease. J Hepatol 2021;75:810-9. [Crossref] [PubMed]
- Derynck R, Akhurst RJ, Balmain A. TGF-beta signaling in tumor suppression and cancer progression. Nat Genet 2001;29:117-29. [Crossref] [PubMed]
- Heldin CH, Landström M, Moustakas A. Mechanism of TGF-beta signaling to growth arrest, apoptosis, and epithelial-mesenchymal transition. Curr Opin Cell Biol 2009;21:166-76. [Crossref] [PubMed]
- Zhang X, Yu WP, Gao L, et al. Effects of lipopolysaccharides stimulated Kupffer cells on activation of rat hepatic stellate cells. World J Gastroenterol 2004;10:610-3. [Crossref] [PubMed]
- Enomoto N, Ikejima K, Bradford B, et al. Alcohol causes both tolerance and sensitization of rat Kupffer cells via mechanisms dependent on endotoxin. Gastroenterology 1998;115:443-51. [Crossref] [PubMed]
- Whittaker S, Marais R, Zhu AX. The role of signaling pathways in the development and treatment of hepatocellular carcinoma. Oncogene 2010;29:4989-5005. [Crossref] [PubMed]
- Turner N, Grose R. Fibroblast growth factor signalling: from development to cancer. Nat Rev Cancer 2010;10:116-29. [Crossref] [PubMed]
- Knights V, Cook SJ. De-regulated FGF receptors as therapeutic targets in cancer. Pharmacol Ther 2010;125:105-17. [Crossref] [PubMed]
- Machida K, Tsukamoto H, Mkrtchyan H, et al. Toll-like receptor 4 mediates synergism between alcohol and HCV in hepatic oncogenesis involving stem cell marker Nanog. Proc Natl Acad Sci U S A 2009;106:1548-53. [Crossref] [PubMed]
- Chen CL, Tsukamoto H, Liu JC, et al. Reciprocal regulation by TLR4 and TGF-ß in tumor-initiating stem-like cells. J Clin Invest 2013;123:2832-49. [Crossref] [PubMed]
- Coulouarn C, Clément B. Stellate cells and the development of liver cancer: therapeutic potential of targeting the stroma. J Hepatol 2014;60:1306-9. [Crossref] [PubMed]
- Obe G, Jonas R, Schmidt S. Metabolism of ethanol in vitro produces a compound which induces sister-chromatid exchanges in human peripheral lymphocytes in vitro: acetaldehyde not ethanol is mutagenic. Mutat Res 1986;174:47-51. [Crossref] [PubMed]
- Helander A, Lindahl-Kiessling K. Increased frequency of acetaldehyde-induced sister-chromatid exchanges in human lymphocytes treated with an aldehyde dehydrogenase inhibitor. Mutat Res 1991;264:103-7. [Crossref] [PubMed]
- Seitz HK, Stickel F. Acetaldehyde as an underestimated risk factor for cancer development: role of genetics in ethanol metabolism. Genes Nutr 2010;5:121-8. [Crossref] [PubMed]
- Fang JL, Vaca CE. Detection of DNA adducts of acetaldehyde in peripheral white blood cells of alcohol abusers. Carcinogenesis 1997;18:627-32. [Crossref] [PubMed]
- Wang M, McIntee EJ, Cheng G, et al. Identification of DNA adducts of acetaldehyde. Chem Res Toxicol 2000;13:1149-57. [Crossref] [PubMed]
- Theruvathu JA, Jaruga P, Nath RG, et al. Polyamines stimulate the formation of mutagenic 1,N2-propanodeoxyguanosine adducts from acetaldehyde. Nucleic Acids Res 2005;33:3513-20. [Crossref] [PubMed]
- Niemelä O. Distribution of ethanol-induced protein adducts in vivo: relationship to tissue injury. Free Radic Biol Med 2001;31:1533-8. [Crossref] [PubMed]
- Espina N, Lima V, Lieber CS, et al. In vitro and in vivo inhibitory effect of ethanol and acetaldehyde on O6-methylguanine transferase. Carcinogenesis 1988;9:761-6. [Crossref] [PubMed]
- Stickel F, Herold C, Seitz HK, et al. Alcohol and methyl transfer: implications for alcohol related hepatocarcinogenesis. In: Ali S, Friedman SL, Mann DA. editors. Liver diseases: biochemical mechanisms and new therapeutic insights. Science Publishers, Enfield/Jersey/Plymouth. 2006:45-58.
- Nieto N, Friedman SL, Cederbaum AI. Cytochrome P450 2E1-derived reactive oxygen species mediate paracrine stimulation of collagen I protein synthesis by hepatic stellate cells. J Biol Chem 2002;277:9853-64. [Crossref] [PubMed]
- Seitz HK, Wang XD. The role of Cytochrome P4502E1 in ethanol mediated carcinogenesis. In: Cytochrome P4502E1: its role in disease and drug metabolism (ed). A Dey Springer Dordrechts, Heidelberg, New York, London, 2013:131-44.
- Lieber CS, DeCarli LM. Hepatic microsomal ethanol-oxidizing system. In vitro characteristics and adaptive properties in vivo. J Biol Chem 1970;245:2505-12. [Crossref] [PubMed]
- Gebhardt AC, Lucas D, Menez JF, et al. Clomethiazole inhibition of cytochrome P4502E1 as assessed by chlorzoxazone hydroxylation in humans. Hepatology 1997;26:957-61. [Crossref] [PubMed]
- French SW. The importance of CYP2E1 in the pathogenesis of alcoholic liver disease and drug toxicity and the role of the proteasome. Subcell Biochem 2013;67:145-64. [Crossref] [PubMed]
- Oneta CM, Lieber CS, Li J, et al. Dynamics of cytochrome P4502E1 activity in man: induction by ethanol and disappearance during withdrawal phase. J Hepatol 2002;36:47-52. [Crossref] [PubMed]
- Koehler BC, Arslic-Schmitt T, Peccerella T, et al. Possible Mechanisms of Ethanol-Mediated Colorectal Carcinogenesis: The Role of Cytochrome P4502E1, Etheno-DNA Adducts, and the Anti-Apoptotic Protein Mcl-1. Alcohol Clin Exp Res 2016;40:2094-101. [Crossref] [PubMed]
- Forsyth CB, Voigt RM, Keshavarzian A. Intestinal CYP2E1: A mediator of alcohol-induced gut leakiness. Redox Biol 2014;3:40-6. [Crossref] [PubMed]
- Seitz HK, Garro AJ, Lieber CS. Enhanced pulmonary and intestinal activation of procarcinogens and mutagens after chronic ethanol consumption in the rat. Eur J Clin Invest 1981;11:33-8. [Crossref] [PubMed]
- Garro AJ, Seitz HK, Lieber CS. Enhancement of dimethylnitrosamine metabolism and activation to a mutagen following chronic ethanol consumption. Cancer Res 1981;41:120-4. [PubMed]
- Seitz HK, Osswald B. Effect of ethanol on procarcinogen activation. In: Watson RR (ed). Alcohol and cancer. CRC Press, Boca Raton, 1992:55-72.
- Gomm W, Röthlein C, Schüssel K, et al. N-Nitrosodimethylamine-Contaminated Valsartan and the Risk of Cancer. Dtsch Arztebl Int 2021;118:357-62. [Crossref] [PubMed]
- Amelizad S, Appel KE, Schoepke M, et al. Enhanced demethylation and denitrosation of N-nitrosodimethylamne by human liver microsomes from alcoholics. Cancer Lett 1989;46:43-9. [Crossref] [PubMed]
- Leo MA, Lieber CS. Hepatic vitamin A depletion in alcoholic liver injury. N Engl J Med 1982;307:597-601. [Crossref] [PubMed]
- Liu C, Chung J, Seitz HK, et al. Chlormethiazole treatment prevents reduced hepatic vitamin A levels in ethanol-fed rats. Alcohol Clin Exp Res 2002;26:1703-9. [Crossref] [PubMed]
- Chung J, Liu C, Smith DE, et al. Restoration of retinoic acid concentration suppresses ethanol-enhanced c-Jun expression and hepatocyte proliferation in rat liver. Carcinogenesis 2001;22:1213-9. [Crossref] [PubMed]
- Wang XD, Liu C, Chung J, et al. Chronic alcohol intake induces retinoic acid concentration and enhances AP-1 (c-jun and c-fos) expression in rat liver. Hepatology 1998;28:744-50. [Crossref] [PubMed]
- Leo MA, Aleynik SI, Aleynik MK, et al. beta-Carotene beadlets potentiate hepatotoxicity of alcohol. Am J Clin Nutr 1997;66:1461-9. [Crossref] [PubMed]
- Albano E. Alcohol, oxidative stress and free radical damage. Proc Nutr Soc 2006;65:278-90. [Crossref] [PubMed]
- Cederbaum AI. Nrf2 and antioxidant defense against CYP2E1 toxicity. Subcell Biochem 2013;67:105-30. [Crossref] [PubMed]
- el Ghissassi F, Barbin A, Nair J, et al. Formation of 1,N6-ethenoadenine and 3,N4-ethenocytosine by lipid peroxidation products and nucleic acid bases. Chem Res Toxicol 1995;8:278-83. [Crossref] [PubMed]
- Blair IA. DNA adducts with lipid peroxidation products. J Biol Chem 2008;283:15545-9. [Crossref] [PubMed]
- Chung FL, Chen HJ, Nath RG. Lipid peroxidation as a potential endogenous source for the formation of exocyclic DNA adducts. Carcinogenesis 1996;17:2105-11. [Crossref] [PubMed]
- Pandya GA, Moriya M. 1,N6-ethenodeoxyadenosine, a DNA adduct highly mutagenic in mammalian cells. Biochemistry 1996;35:11487-92. [Crossref] [PubMed]
- Levine RL, Yang IY, Hossain M, et al. Mutagenesis induced by a single 1,N6-ethenodeoxyadenosine adduct in human cells. Cancer Res 2000;60:4098-104. [PubMed]
- Hu W, Feng Z, Eveleigh J, et al. The major lipid peroxidation product, trans-4-hydroxy-2-nonenal, preferentially forms DNA adducts at codon 249 of human p53 gene, a unique mutational hotspot in hepatocellular carcinoma. Carcinogenesis 2002;23:1781-9. [Crossref] [PubMed]
- Frank A, Nair J, Seitz HK, et al. Immunohistochemical detection of 1,-N6ethenodeoxyadenosine in nuclei of human livers effected by disease predisposing hepatocarcinogenesis. Carcinogenesis 2004;25:1027-31. [Crossref] [PubMed]
- Linhart K, Bartsch HU, Seitz HK. The role of reactive oxygen species (ROS) and cytochrome P4502E1 in the generation of carcinogenic DNA adducts. Redox Biol 2014;3:56-62. [Crossref] [PubMed]
- Wang Y, Millonig G, Nair J, et al. Ethanol-induced cytochrome P4502E1 causes carcinogenic etheno-DNA lesions in alcoholic liver disease. Hepatology 2009;50:453-61. [Crossref] [PubMed]
- Lu Y, Wu D, Wang X, et al. Chronic alcohol-induced liver injury and oxidant stress are decreased in cytochrome P4502E1 knockout mice and restored in humanized cytochrome P4502E1 knock-in mice. Free Radic Biol Med 2010;49:1406-16. [Crossref] [PubMed]
- Butura A, Nilsson K, Morgan K, et al. The impact of CYP2E1 on the development of alcoholic liver disease as studied in a transgenic mouse model. J Hepatol 2009;50:572-83. [Crossref] [PubMed]
- Morgan K, French SW, Morgan TR. Production of a cytochrome P450 2E1 transgenic mouse and initial evaluation of alcoholic liver damage. Hepatology 2002;36:122-34. [Crossref] [PubMed]
- Gouillon Z, Lucas D, Li J, et al. Inhibition of ethanol-induced liver disease in the intragastric feeding rat model by chlormethiazole. Proc Soc Exp Biol Med 2000;224:302-8. [Crossref] [PubMed]
- Ye Q, Lian F, Chavez PR, et al. Cytochrome P450 2E1 inhibition prevents hepatic carcinogenesis induced by diethylnitrosamine in alcohol-fed rats. Hepatobiliary Surg Nutr 2012;1:5-18. [PubMed]
- Chavez PR, Lian F, Chung J, et al. Long-term ethanol consumption promotes hepatic tumorigenesis but impairs normal hepatocyte proliferation in rats. J Nutr 2011;141:1049-55. [Crossref] [PubMed]
- Mercer KE, Hennings L, Sharma N, et al. Alcohol consumption promotes diethylnitrosamine-induced hepatocarcinogenesis in male mice through activation of the Wnt/β-catenin signaling pathway. Cancer Prev Res (Phila) 2014;7:675-85. [Crossref] [PubMed]
- Groll N, Petrikat T, Vetter S, et al. Coordinate regulation of CYP2E1 by ß-catenine and hepatocyte nuclear factor 1a-dependent signaling. Toxicology 2016;350-352:40-8. [Crossref] [PubMed]
- Mueller S, Peccerella T, Qin H, et al. Carcinogenic Etheno DNA Adducts in Alcoholic Liver Disease: Correlation with Cytochrome P-4502E1 and Fibrosis. Alcohol Clin Exp Res 2018;42:252-9. [Crossref] [PubMed]
- Hohmann N, Schröder F, Moreira B, et al. Clomethiazole inhibits cytochrome P450 2E1 and improves alcoholic liver disease. Gut 2022;71:842-44. [Crossref] [PubMed]
- Ganne-Carrié N, Christidis C, Chastang C, et al. Liver iron is predictive of death in alcoholic cirrhosis: a multivariate study of 229 consecutive patients with alcoholic and/or hepatitis C virus cirrhosis: a prospective follow up study. Gut 2000;46:277-82. [Crossref] [PubMed]
- Ribot-Hernández I, Martín-González C, Vera-Delgado V, et al. Prognostic Value of Serum Iron, Ferritin, and Transferrin in Chronic Alcoholic Liver Disease. Biol Trace Elem Res 2020;195:427-35. [Crossref] [PubMed]
- French SW. Epigenetic events in liver cancer resulting from alcoholic liver disease. Alcohol Res 2013;35:57-67. [PubMed]
- D’Addario C, Maccarrone M. Alcohol and Epigenetic modulations. In: Molecular aspects of alcohol and nutrition (ed. Patel VB). Academic Press, Elesevier London, San Diego, Waltham Oxford, 2016:261-74.
- Resendiz M, Chiao-Ling S, Badin JK, et al. Alcohol metabolism and epigenetic methylation and acetylation. In: Molecular aspects of alcohol and nutrition (ed. Patel VB). Academic Press, Elesevier London, San Diego, Waltham Oxford, 2016:261-74.
- Hitchler MJ, Domann FE. Metabolic defects provide a spark for the epigenetic switch in cancer. Free Radic Biol Med 2009;47:115-27. [Crossref] [PubMed]
- Weitzman SA, Turk PW, Milkowski DH, et al. Free radical adducts induce alterations in DNA cytosine methylation. Proc Natl Acad Sci U S A 1994;91:1261-4. [Crossref] [PubMed]
- Bardag-Gorce F, French BA, Joyce M, et al. Histone acetyltransferase p300 modulates gene expression in an epigenetic manner at high blood alcohol levels. Exp Mol Pathol 2007;82:197-202. [Crossref] [PubMed]
- Dokmanovic M, Clarke C, Marks PA. Histone deacetylase inhibitors: overview and perspectives. Mol Cancer Res 2007;5:981-9. [Crossref] [PubMed]
- Gui CY, Ngo L, Xu WS, et al. Histone deacetylase (HDAC) inhibitor activation of p21WAF1 involves changes in promoter-associated proteins, including HDAC1. Proc Natl Acad Sci U S A 2004;101:1241-6. [Crossref] [PubMed]
- Maeda M, Nagawa H, Maeda T, et al. Alcohol consumption enhances liver metastasis in colorectal carcinoma patients. Cancer 1998;83:1483-8. [Crossref] [PubMed]
- Yan G, Wang X, Sun C, et al. Chronic Alcohol Consumption Promotes Diethylnitrosamine-Induced Hepatocarcinogenesis via Immune Disturbances. Sci Rep 2017;7:2567. [Crossref] [PubMed]
- Task Force on Community Preventive Services. Recommendations on dram shop liability and overservice law enforcement initiatives to prevent excessive alcohol consumption and related harms. Am J Prev Med 2011;41:344-6. [Crossref] [PubMed]
Cite this article as: Seitz HK, Neuman M. Narrative review on alcoholic liver disease: from fibrosis to cancer. Dig Med Res 2022;5:15.